Health
Translational molecular imaging and drug development in Parkinson’s disease | Molecular Neurodegeneration
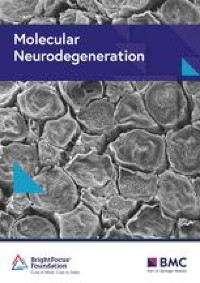
Bloem BR, Okun MS, Klein C. Parkinson’s disease. The Lancet. 2021;397:2284–303.
Ovallath S, Deepa P. The history of parkinsonism: descriptions in ancient Indian medical literature. Mov Disord. 2013;28:566–8.
Parkinson J. An Essay on the Shaking Palsy. 1817. J Neuropsychiatry Clin Neurosci. 2002;14:223–36.
Goetz CG. The history of Parkinson’s disease: early clinical descriptions and neurological therapies. Cold Spring Harb Perspect Med. 2011;1:a008862–a008862.
Nussbaum RL, Ellis CE. Alzheimer’s disease and Parkinson’s disease. N Engl J Med. 2003;348:1356–64.
Collaborators GN. Global, regional, and national burden of neurological disorders, 1990–2016: a systematic analysis for the Global Burden of Disease Study 2016. Lancet Neurol. 2019;18:459–80.
Papapetropoulos S, Adi N, Ellul J, Argyriou AA, Chroni E. A prospective study of familial versus sporadic Parkinson’s disease. Neurodegener Dis. 2007;4:424–7.
Braak H, Ghebremedhin E, Rüb U, Bratzke H, Del Tredici K. Stages in the development of Parkinson’s disease-related pathology. Cell Tissue Res. 2004;318:121–34.
Masato A, Plotegher N, Boassa D, Bubacco L. Impaired dopamine metabolism in Parkinson’s disease pathogenesis. Mol Neurodegener. 2019;14:35.
Sveinbjornsdottir S. The clinical symptoms of Parkinson’s disease. J Neurochem. 2016;139:318–24.
Cheng HC, Ulane CM, Burke RE. Clinical progression in Parkinson disease and the neurobiology of axons. Ann Neurol. 2010;67:715–25.
Braak H, Bohl JR, Müller CM, Rüb U, de Vos RAI, Del Tredici K. Stanley Fahn Lecture 2005: The staging procedure for the inclusion body pathology associated with sporadic Parkinson’s disease reconsidered. Mov Disord. 2006;21:2042–51.
Poewe W, Seppi K, Tanner CM, Halliday GM, Brundin P, Volkmann J, Schrag A-E, Lang AE. Parkinson disease. Nat Rev Dis Primers. 2017;3:17013.
Jankovic J. Parkinson’s disease: clinical features and diagnosis. J Neurol Neurosurg Psychiatry. 2008;79:368–76.
Solari N, Bonito-Oliva A, Fisone G, Brambilla R. Understanding cognitive deficits in Parkinson’s disease: lessons from preclinical animal models. Learn Mem. 2013;20:592–600.
Schneider F, Althaus A, Backes V, Dodel R. Psychiatric symptoms in Parkinson’s disease. Eur Arch Psychiatry Clin Neurosci. 2008;258:55–9.
Pagano G, Niccolini F, Politis M. Imaging in Parkinson’s disease Clin Med (Lond). 2016;16:371–5.
Schapira AHV, Gu M, Taanman JW, Tabrizi SJ, Seaton T, Cleeter M, Cooper JM. Mitochondria in the etiology and pathogenesis of parkinson’s disease. Ann Neurol. 1998;44:S89–98.
Park J-S, Davis RL, Sue CM. Mitochondrial Dysfunction in Parkinson’s Disease: New Mechanistic Insights and Therapeutic Perspectives. Curr Neurol Neurosci Rep. 2018;18(5):21.
Jenner P, Olanow CW. Oxidative stress and the pathogenesis of Parkinson’s disease. Neurology. 1996;47:161S.
Blesa J, Trigo-Damas I, Quiroga-Varela A, Jackson-Lewis VR. Oxidative stress and Parkinson’s disease. Front Neuroanatomy. 2015;9:91.
Hirsch EC, Hunot S. Neuroinflammation in Parkinson’s disease: a target for neuroprotection? The Lancet Neurology. 2009;8:382–97.
Wang Q, Liu Y, Zhou J. Neuroinflammation in Parkinson’s disease and its potential as therapeutic target. Transl Neurodegener. 2015;4:19.
Ambrosi G, Cerri S, Blandini F. A further update on the role of excitotoxicity in the pathogenesis of Parkinson’s disease. J Neural Transm (Vienna, Austria : 1996). 2014;121:849–59.
van der Brug MP, Singleton A, Gasser T, Lewis PA. Parkinson’s disease: From human genetics to clinical trials. Sci Transl Med. 2015;7:205ps220.
Funayama M, Nishioka K, Li Y, Hattori N. Molecular genetics of Parkinson’s disease: Contributions and global trends. J Hum Genet. 2022. https://doi.org/10.1038/s10038-022-01058-5.
McNaught KS, Olanow CW. Protein aggregation in the pathogenesis of familial and sporadic Parkinson’s disease. Neurobiol Aging. 2006;27:530–45.
Akhtar RS, Xie SX, Brennan L, Pontecorvo MJ, Hurtig HI, Trojanowski JQ, Weintraub D, Siderowf AD. Amyloid-Beta Positron Emission Tomography Imaging of Alzheimer’s Pathology in Parkinson’s Disease Dementia. Mov Disord Clin Pract. 2016;3:367–75.
Murphy MP, Bayir H, Belousov V, Chang CJ, Davies KJA, Davies MJ, Dick TP, Finkel T, Forman HJ, Janssen-Heininger Y, et al. Guidelines for measuring reactive oxygen species and oxidative damage in cells and in vivo. Nat Metab. 2022;4:651–62.
Chaudhuri KR, Schapira AH. Non-motor symptoms of Parkinson’s disease: dopaminergic pathophysiology and treatment. Lancet Neurol. 2009;8:464–74.
Braak H, Braak E. Pathoanatomy of Parkinson’s disease. J Neurol. 2000;247:II3–10.
Rascol O, Payoux P, Ory F, Ferreira JJ, Brefel-Courbon C, Montastruc JL. Limitations of current Parkinson’s disease therapy. Ann Neurol. 2003;53(Suppl 3):S3-12; discussion S12-5.
Armstrong MJ, Okun MS. Diagnosis and Treatment of Parkinson Disease: A Review. JAMA. 2020;323:548–60.
Verger A, Grimaldi S, Ribeiro MJ, Frismand S, Guedj E. Single Photon Emission Computed Tomography/Positron Emission Tomography Molecular Imaging for Parkinsonism: A Fast-Developing Field. Ann Neurol. 2021;90:711–9.
Nerella SG, Singh P, Sanam T, Digwal CS. PET Molecular Imaging in Drug Development: The Imaging and Chemistry Perspective. Front Med (Lausanne). 2022;9:812270.
Lu FM, Yuan Z. PET/SPECT molecular imaging in clinical neuroscience: recent advances in the investigation of CNS diseases. Quant Imaging Med Surg. 2015;5:433–47.
Ametamey SM, Honer M, Schubiger PA. Molecular imaging with PET. Chem Rev. 2008;108:1501–16.
Zhu L, Ploessl K, Kung HF. PET/SPECT imaging agents for neurodegenerative diseases. Chem Soc Rev. 2014;43:6683–91.
Pavese N, Brooks DJ. Imaging neurodegeneration in Parkinson’s disease. Biochem Biophys Acta. 2009;1792:722–9.
Matthews DC, Lerman H, Lukic A, Andrews RD, Mirelman A, Wernick MN, Giladi N, Strother SC, Evans KC, Cedarbaum JM, Even-Sapir E. FDG PET Parkinson’s disease-related pattern as a biomarker for clinical trials in early stage disease. Neuroimage Clin. 2018;20:572–9.
Meyer PT, Frings L, Rücker G, Hellwig S. (18)F-FDG PET in Parkinsonism: Differential Diagnosis and Evaluation of Cognitive Impairment. J Nucl Med. 2017;58:1888–98.
Garraux G, Phillips C, Schrouff J, Kreisler A, Lemaire C, Degueldre C, Delcour C, Hustinx R, Luxen A, Destée A, Salmon E. Multiclass classification of FDG PET scans for the distinction between Parkinson’s disease and atypical parkinsonian syndromes. Neuroimage Clin. 2013;2:883–93.
Mudali D, Teune LK, Renken RJ, Leenders KL, Roerdink JB. Classification of Parkinsonian syndromes from FDG-PET brain data using decision trees with SSM/PCA features. Comput Math Methods Med. 2015;2015:136921.
Nalls MA, Pankratz N, Lill CM, Do CB, Hernandez DG, Saad M, DeStefano AL, Kara E, Bras J, Sharma M, et al. Large-scale meta-analysis of genome-wide association data identifies six new risk loci for Parkinson’s disease. Nat Genet. 2014;46:989–93.
Wassouf Z, Schulze-Hentrich JM. Alpha-synuclein at the nexus of genes and environment: the impact of environmental enrichment and stress on brain health and disease. J Neurochem. 2019;150:591–604.
Goedert M. Alpha-synuclein and neurodegenerative diseases. Nat Rev Neurosci. 2001;2:492–501.
Vekrellis K, Xilouri M, Emmanouilidou E, Rideout HJ, Stefanis L. Pathological roles of α-synuclein in neurological disorders. Lancet Neurol. 2011;10:1015–25.
Spillantini MG, Schmidt ML, Lee VM, Trojanowski JQ, Jakes R, Goedert M. Alpha-synuclein in Lewy bodies. Nature. 1997;388:839–40.
Wakabayashi K, Tanji K, Odagiri S, Miki Y, Mori F, Takahashi H. The Lewy Body in Parkinson’s Disease and Related Neurodegenerative Disorders. Mol Neurobiol. 2013;47:495–508.
Benskey MJ, Perez RG, Manfredsson FP. The contribution of alpha synuclein to neuronal survival and function – Implications for Parkinson’s disease. J Neurochem. 2016;137:331–59.
Polymeropoulos MH. Mutation in the -Synuclein Gene Identified in Families with Parkinson’s Disease. Science. 1997;276:2045–7.
Kruger R, Kuhn W, Muller T, Woitalla D, Graeber M, Kosel S, Przuntek H, Epplen JT, Schols L, Riess O. Ala30Pro mutation in the gene encoding alpha-synuclein in Parkinson’s disease. Nat Genet. 1998;18:106–8.
Zarranz JJ, Alegre J, Gómez-Esteban JC, Lezcano E, Ros R, Ampuero I, Vidal L, Hoenicka J, Rodriguez O, Atarés B, et al. The new mutation, E46K, of α-synuclein causes parkinson and Lewy body dementia. Ann Neurol. 2004;55:164–73.
Appel-Cresswell S, Vilarino-Guell C, Encarnacion M, Sherman H, Yu I, Shah B, Weir D, Thompson C, Szu-Tu C, Trinh J, et al. Alpha-synuclein p.H50Q, a novel pathogenic mutation for Parkinson’s disease. Mov Disord. 2013;28:811–3.
Kiely AP, Asi YT, Kara E, Limousin P, Ling H, Lewis P, Proukakis C, Quinn N, Lees AJ, Hardy J, et al. α-Synucleinopathy associated with G51D SNCA mutation: a link between Parkinson’s disease and multiple system atrophy? Acta Neuropathol. 2013;125:753–69.
Pasanen P, Myllykangas L, Siitonen M, Raunio A, Kaakkola S, Lyytinen J, Tienari PJ, Pöyhönen M, Paetau A. A novel α-synuclein mutation A53E associated with atypical multiple system atrophy and Parkinson’s disease-type pathology. Neurobiol Aging. 2014;35:2180.e2181-2180.e2185.
Lesage S, Anheim M, Letournel F, Bousset L, Honoré A, Rozas N, Pieri L, Madiona K, Dürr A, Melki R, et al. G51D α-synuclein mutation causes a novel Parkinsonian-pyramidal syndrome. Ann Neurol. 2013;73:459–71.
Proukakis C, Dudzik CG, Brier T, MacKay DS, Cooper JM, Millhauser GL, Houlden H, Schapira AH. A novel α-synuclein missense mutation in Parkinson disease. Neurology. 2013;80:1062.
Phillipson OT. Alpha-synuclein, epigenetics, mitochondria, metabolism, calcium traffic, & circadian dysfunction in Parkinson’s disease. An integrated strategy for management. Ageing Res Rev. 2017;40:149–67.
Yoshino H, Hirano M, Stoessl AJ, Imamichi Y, Ikeda A, Li Y, Funayama M, Yamada I, Nakamura Y, Sossi V, et al. Homozygous alpha-synuclein p.A53V in familial Parkinson’s disease. Neurobiol Aging. 2017;57(248):e247-248 e212.
Chavarría C, Souza JM. Oxidation and nitration of α-synuclein and their implications in neurodegenerative diseases. Arch Biochem Biophys. 2013;533:25–32.
Shimura H, Schlossmacher MG, Hattori N, Frosch MP, Trockenbacher A, Schneider R, Mizuno Y, Kosik KS, Selkoe DJ. Ubiquitination of a new form of alpha-synuclein by parkin from human brain: implications for Parkinson’s disease. Science. 2001;293:263–9.
Paleologou KE, Oueslati A, Shakked G, Rospigliosi CC, Kim HY, Lamberto GR, Fernandez CO, Schmid A, Chegini F, Gai WP, et al. Phosphorylation at S87 Is Enhanced in Synucleinopathies, Inhibits -Synuclein Oligomerization, and Influences Synuclein-Membrane Interactions. J Neurosci. 2010;30:3184–98.
Shah M, Seibyl J, Cartier A, Bhatt R, Catafau AM. Molecular imaging insights into neurodegeneration: focus on alpha-synuclein radiotracers. J Nucl Med. 2014;55:1397–400.
Ye L, Velasco A, Fraser G, Beach TG, Sue L, Osredkar T, Libri V, Spillantini MG, Goedert M, Lockhart A. In vitro high affinity alpha-synuclein binding sites for the amyloid imaging agent PIB are not matched by binding to Lewy bodies in postmortem human brain. J Neurochem. 2008;105:1428–37.
Fodero-Tavoletti MT, Smith DP, McLean CA, Adlard PA, Barnham KJ, Foster LE, Leone L, Perez K, Cortes M, Culvenor JG, et al. In vitro characterization of Pittsburgh compound-B binding to Lewy bodies. J Neurosci. 2007;27:10365–71.
Levigoureux E, Lancelot S, Bouillot C, Chauveau F, Verdurand M, Verchere J, Billard T, Baron T, Zimmer L. Binding of the PET radiotracer [(1)(8)F]BF227 does not reflect the presence of alpha-synuclein aggregates in transgenic mice. Curr Alzheimer Res. 2014;11:955–60.
Fodero-Tavoletti MT, Mulligan RS, Okamura N, Furumoto S, Rowe CC, Kudo Y, Masters CL, Cappai R, Yanai K, Villemagne VL. In vitro characterisation of BF227 binding to alpha-synuclein/Lewy bodies. Eur J Pharmacol. 2009;617:54–8.
Yu L, Cui J, Padakanti PK, Engel L, Bagchi DP, Kotzbauer PT, Tu Z. Synthesis and in vitro evaluation of alpha-synuclein ligands. Bioorg Med Chem. 2012;20:4625–34.
Bagchi DP, Yu L, Perlmutter JS, Xu J, Mach RH, Tu Z, Kotzbauer PT. Binding of the Radioligand SIL23 to α-Synuclein Fibrils in Parkinson Disease Brain Tissue Establishes Feasibility and Screening Approaches for Developing a Parkinson Disease Imaging Agent. PLoS ONE. 2013;8(2):e55031.
Zhang X, Jin H, Padakanti P, Li J, Yang H, Fan J, Mach R, Kotzbauer P, Tu Z. Radiosynthesis and in Vivo Evaluation of Two PET Radioligands for Imaging α-Synuclein. Appl Sci. 2014;4(1):66–78.
Ferrie JJ, Lengyel-Zhand Z, Janssen B, Lougee MG, Giannakoulias S, Hsieh CJ, Pagar VV, Weng CC, Xu H, Graham TJA, et al. Identification of a nanomolar affinity alpha-synuclein fibril imaging probe by ultra-high throughput in silico screening. Chem Sci. 2020;11:12746–54.
Miranda-Azpiazu P, Svedberg M, Higuchi M, Ono M, Jia Z, Sunnemark D, Elmore CS, Schou M, Varrone A. Identification and in vitro characterization of C05–01, a PBB3 derivative with improved affinity for alpha-synuclein. Brain Res. 2020;1749:147131.
Chen YF, Bian J, Zhang P, Bu LL, Shen Y, Yu WB, Lu XH, Lin X, Ye DY, Wang J, Chu Y. Design, synthesis and identification of N, N-dibenzylcinnamamide (DBC) derivatives as novel ligands for alpha-synuclein fibrils by SPR evaluation system. Bioorg Med Chem. 2020;28:115358.
Kaide S, Watanabe H, Shimizu Y, Iikuni S, Nakamoto Y, Hasegawa M, Itoh K, Ono M. Identification and Evaluation of Bisquinoline Scaffold as a New Candidate for alpha-Synuclein-PET Imaging. ACS Chem Neurosci. 2020;11:4254–61.
Kuebler L, Buss S, Leonov A, Ryazanov S, Schmidt F, Maurer A, Weckbecker D, Landau AM, Lillethorup TP, Bleher D, et al. [(11)C]MODAG-001-towards a PET tracer targeting alpha-synuclein aggregates. Eur J Nucl Med Mol Imaging. 2021;48:1759–72.
Matsuoka K, Ono M, Takado Y, Hirata K, Endo H, Ohfusa T, Kojima T, Yamamoto T, Onishi T, Orihara A, et al. High-Contrast Imaging of α-Synuclein Pathologies in Living Patients with Multiple System Atrophy. Movement Disorders. 2022;37(10):2159–61. https://doi.org/10.1002/mds.29186.
Capotosti F, Vokali E, Molette J, Ravache M, Delgado C, Kocher J, Pittet L, Dimitrakopoulos IK, Di-Bonaventura I, Touilloux T, et al. The development of [18F]ACI-12589, a high affinity and selective alpha-synuclein radiotracer, as a biomarker for Parkinson’s disease and other synucleinopathies. Alzheimers Dement. 2021;17:e053943.
Roshanbin S, Xiong M, Hultqvist G, Söderberg L, Zachrisson O, Meier S, Ekmark-Lewén S, Bergström J, Ingelsson M, Sehlin D, Syvänen S. In vivo imaging of alpha-synuclein with antibody-based PET. Neuropharmacology. 2022;208:108985.
Fujiwara H, Hasegawa M, Dohmae N, Kawashima A, Masliah E, Goldberg MS, Shen J, Takio K, Iwatsubo T. alpha-Synuclein is phosphorylated in synucleinopathy lesions. Nat Cell Biol. 2002;4:160–4.
Irwin DJ, Lee VM, Trojanowski JQ. Parkinson’s disease dementia: convergence of α-synuclein, tau and amyloid-β pathologies. Nat Rev Neurosci. 2013;14:626–36.
Van der Perren A, Gelders G, Fenyi A, Bousset L, Brito F, Peelaerts W, Van den Haute C, Gentleman S, Melki R, Baekelandt V. The structural differences between patient-derived α-synuclein strains dictate characteristics of Parkinson’s disease, multiple system atrophy and dementia with Lewy bodies. Acta Neuropathol. 2020;139:977–1000.
McKeith IG, Galasko D, Kosaka K, Perry EK, Dickson DW, Hansen LA, Salmon DP, Lowe J, Mirra SS, Byrne EJ, et al. Consensus guidelines for the clinical and pathologic diagnosis of dementia with Lewy bodies (DLB): report of the consortium on DLB international workshop. Neurology. 1996;47:1113–24.
Lippa CF, Duda JE, Grossman M, Hurtig HI, Aarsland D, Boeve BF, Brooks DJ, Dickson DW, Dubois B, Emre M, et al. DLB and PDD boundary issues: diagnosis, treatment, molecular pathology, and biomarkers. Neurology. 2007;68:812–9.
van Rumund A, Green AJE, Fairfoul G, Esselink RAJ, Bloem BR, Verbeek MM. α-Synuclein real-time quaking-induced conversion in the cerebrospinal fluid of uncertain cases of parkinsonism. Ann Neurol. 2019;85:777–81.
Iranzo A, Fairfoul G, Ayudhaya ACN, Serradell M, Gelpi E, Vilaseca I, Sanchez-Valle R, Gaig C, Santamaria J, Tolosa E, et al. Detection of α-synuclein in CSF by RT-QuIC in patients with isolated rapid-eye-movement sleep behaviour disorder: a longitudinal observational study. Lancet Neurol. 2021;20:203–12.
Lee JM, Derkinderen P, Kordower JH, Freeman R, Munoz DG, Kremer T, Zago W, Hutten SJ, Adler CH, Serrano GE, Beach TG. The Search for a Peripheral Biopsy Indicator of α-Synuclein Pathology for Parkinson Disease. J Neuropathol Exp Neurol. 2017;76:2–15.
Antelmi E, Donadio V, Incensi A, Plazzi G, Liguori R. Skin nerve phosphorylated α-synuclein deposits in idiopathic REM sleep behavior disorder. Neurology. 2017;88:2128–31.
Donadio V, Incensi A, Piccinini C, Cortelli P, Giannoccaro MP, Baruzzi A, Liguori R. Skin nerve misfolded α-synuclein in pure autonomic failure and Parkinson disease. Ann Neurol. 2016;79:306–16.
Donadio V, Incensi A, Rizzo G, Capellari S, Pantieri R, Stanzani Maserati M, Devigili G, Eleopra R, Defazio G, Montini F, et al. A new potential biomarker for dementia with Lewy bodies: Skin nerve α-synuclein deposits. Neurology. 2017;89:318–26.
Gibbons CH, Garcia J, Wang N, Shih LC, Freeman R. The diagnostic discrimination of cutaneous α-synuclein deposition in Parkinson disease. Neurology. 2016;87:505–12.
Visanji NP, Mollenhauer B, Beach TG, Adler CH, Coffey CS, Kopil CM, Dave KD, Foroud T, Chahine L, Jennings D. The Systemic Synuclein Sampling Study: toward a biomarker for Parkinson’s disease. Biomark Med. 2017;11:359–68.
Wang Z, Becker K, Donadio V, Siedlak S, Yuan J, Rezaee M, Incensi A, Kuzkina A, Orrú CD, Tatsuoka C, et al. Skin α-Synuclein Aggregation Seeding Activity as a Novel Biomarker for Parkinson Disease. JAMA Neurol. 2020;78:1–11.
Donadio V, Doppler K, Incensi A, Kuzkina A, Janzen A, Mayer G, Volkmann J, Rizzo G, Antelmi E, Plazzi G, et al. Abnormal α-synuclein deposits in skin nerves: intra- and inter-laboratory reproducibility. Eur J Neurol. 2019;26:1245–51.
Fields CR, Bengoa-Vergniory N, Wade-Martins R. Targeting Alpha-Synuclein as a Therapy for Parkinson’s Disease. Front Mol Neurosci. 2019;12:299–299.
Sapru MK, Yates JW, Hogan S, Jiang L, Halter J, Bohn MC. Silencing of human alpha-synuclein in vitro and in rat brain using lentiviral-mediated RNAi. Exp Neurol. 2006;198:382–90.
Takahashi M, Suzuki M, Fukuoka M, Fujikake N, Watanabe S, Murata M, Wada K, Nagai Y, Hohjoh H. Normalization of Overexpressed α-Synuclein Causing Parkinson’s Disease By a Moderate Gene Silencing With RNA Interference. Molecular Therapy – Nucleic Acids. 2015;4:e241.
Lewis J, Melrose H, Bumcrot D, Hope A, Zehr C, Lincoln S, Braithwaite A, He Z, Ogholikhan S, Hinkle K, et al. In vivo silencing of alpha-synuclein using naked siRNA. Mol Neurodegener. 2008;3:19.
Mittal S, Bjørnevik K, Im DS, Flierl A, Dong X, Locascio JJ, Abo KM, Long E, Jin M, Xu B, et al. β2-Adrenoreceptor is a regulator of the α-synuclein gene driving risk of Parkinson’s disease. Science. 2017;357:891–8.
Klucken J, Shin Y, Masliah E, Hyman BT, McLean PJ. Hsp70 Reduces alpha-Synuclein Aggregation and Toxicity. J Biol Chem. 2004;279:25497–502.
Cox D, Selig E, Griffin MDW, Carver JA, Ecroyd H. Small Heat-shock Proteins Prevent α-Synuclein Aggregation via Transient Interactions and Their Efficacy Is Affected by the Rate of Aggregation. J Biol Chem. 2016;291:22618–29.
Ghochikyan A, Petrushina I, Davtyan H, Hovakimyan A, Saing T, Davtyan A, Cribbs DH, Agadjanyan MG. Immunogenicity of epitope vaccines targeting different B cell antigenic determinants of human α-synuclein: Feasibility study. Neurosci Lett. 2014;560:86–91.
Masliah E, Rockenstein E, Adame A, Alford M, Crews L, Hashimoto M, Seubert P, Lee M, Goldstein J, Chilcote T, et al. Effects of alpha-synuclein immunization in a mouse model of Parkinson’s disease. Neuron. 2005;46:857–68.
Sanchez-Guajardo V, Annibali A, Jensen PH, Romero-Ramos M. alpha-Synuclein vaccination prevents the accumulation of parkinson disease-like pathologic inclusions in striatum in association with regulatory T cell recruitment in a rat model. J Neuropathol Exp Neurol. 2013;72:624–45.
Masliah E, Rockenstein E, Mante M, Crews L, Spencer B, Adame A, Patrick C, Trejo M, Ubhi K, Rohn TT, et al. Passive immunization reduces behavioral and neuropathological deficits in an alpha-synuclein transgenic model of Lewy body disease. PLoS ONE. 2011;6:e19338.
Schenk DB, Koller M, Ness DK, Griffith SG, Grundman M, Zago W, Soto J, Atiee G, Ostrowitzki S, Kinney GG. First-in-human assessment of PRX002, an anti-alpha-synuclein monoclonal antibody, in healthy volunteers. Mov Disord. 2017;32:211–8.
Pagano G, Taylor KI, Anzures-Cabrera J, Marchesi M, Simuni T, Marek K, Postuma RB, Pavese N, Stocchi F, Azulay JP, et al. Trial of Prasinezumab in Early-Stage Parkinson’s Disease. N Engl J Med. 2022;387:421–32.
Fjord-Larsen L, Thougaard A, Wegener KM, Christiansen J, Larsen F, Schrøder-Hansen LM, Kaarde M, Ditlevsen DK. Nonclinical safety evaluation, pharmacokinetics, and target engagement of Lu AF82422, a monoclonal IgG1 antibody against alpha-synuclein in development for treatment of synucleinopathies. MAbs. 2021;13:1994690.
Sevigny J, Chiao P, Bussière T, Weinreb PH, Williams L, Maier M, Dunstan R, Salloway S, Chen T, Ling Y, et al. The antibody aducanumab reduces Aβ plaques in Alzheimer’s disease. Nature. 2016;537:50–6.
van Dyck CH, Swanson CJ, Aisen P, Bateman RJ, Chen C, Gee M, Kanekiyo M, Li D, Reyderman L, Cohen S, et al. Lecanemab in Early Alzheimer’s Disease. N Engl J Med. 2023;388:9–21.
Weihofen A, Liu Y, Arndt JW, Huy C, Quan C, Smith BA, Baeriswyl JL, Cavegn N, Senn L, Su L, et al. Development of an aggregate-selective, human-derived α-synuclein antibody BIIB054 that ameliorates disease phenotypes in Parkinson’s disease models. Neurobiol Dis. 2019;124:276–88.
Lindström V, Fagerqvist T, Nordström E, Eriksson F, Lord A, Tucker S, Andersson J, Johannesson M, Schell H, Kahle PJ, et al. Immunotherapy targeting α-synuclein protofibrils reduced pathology in (Thy-1)-h[A30P] α-synuclein mice. Neurobiol Dis. 2014;69:134–43.
Nordström E, Eriksson F, Sigvardson J, Johannesson M, Kasrayan A, Jones-Kostalla M, Appelkvist P, Söderberg L, Nygren P, Blom M, et al. ABBV-0805, a novel antibody selective for soluble aggregated α-synuclein, prolongs lifespan and prevents buildup of α-synuclein pathology in mouse models of Parkinson’s disease. Neurobiol Dis. 2021;161:105543.
Chen SW, Drakulic S, Deas E, Ouberai M, Aprile FA, Arranz R, Ness S, Roodveldt C, Guilliams T, De-Genst EJ, et al. Structural characterization of toxic oligomers that are kinetically trapped during α-synuclein fibril formation. Proc Natl Acad Sci USA. 2015;112:E1994-2003.
Vaikath NN, Hmila I, Gupta V, Erskine D, Ingelsson M, El-Agnaf OMA. Antibodies against alpha-synuclein: tools and therapies. J Neurochem. 2019;150:612–25.
Kariolis MS, Wells RC, Getz JA, Kwan W, Mahon CS, Tong R, Kim DJ, Srivastava A, Bedard C, Henne KR, et al. Brain delivery of therapeutic proteins using an Fc fragment blood-brain barrier transport vehicle in mice and monkeys. Sci Transl Med. 2020;12(545):eaay1359.
Roshanbin S, Julku U, Xiong M, Eriksson J, Masliah E, Hultqvist G, Bergström J, Ingelsson M, Syvänen S, Sehlin D. Reduction of αSYN Pathology in a Mouse Model of PD Using a Brain-Penetrating Bispecific Antibody. Pharmaceutics. 2022;14(7):1412.
Langston JW, Ballard P, Tetrud JW, Irwin I. Chronic Parkinsonism in humans due to a product of meperidine-analog synthesis. Science. 1983;219:979–80.
Burns RS, Chiueh CC, Markey SP, Ebert MH, Jacobowitz DM, Kopin IJ. A primate model of parkinsonism: selective destruction of dopaminergic neurons in the pars compacta of the substantia nigra by N-methyl-4-phenyl-1,2,3,6-tetrahydropyridine. Proc Natl Acad Sci USA. 1983;80:4546–50.
Langston JW, Forno LS, Rebert CS, Irwin I. Selective nigral toxicity after systemic administration of 1-methyl-4-phenyl-1,2,5,6-tetrahydropyrine (MPTP) in the squirrel monkey. Brain Res. 1984;292:390–4.
Schneider JS, Denaro FJ. Astrocytic responses to the dopaminergic neurotoxin 1-methyl-4-phenyl-1,2,3,6-tetrahydropyridine (MPTP) in cat and mouse brain. J Neuropathol Exp Neurol. 1988;47:452–8.
Sayre LM. Biochemical mechanism of action of the dopaminergic neurotoxin 1-methyl-4-phenyl-1,2,3,6-tetrahydropyridine (MPTP). Toxicol Lett. 1989;48:121–49.
Tipton KF, Singer TP. Advances in our understanding of the mechanisms of the neurotoxicity of MPTP and related compounds. J Neurochem. 1993;61:1191–206.
Schapira AH, Cooper JM, Dexter D, Clark JB, Jenner P, Marsden CD. Mitochondrial complex I deficiency in Parkinson’s disease. J Neurochem. 1990;54:823–7.
Greenamyre JT, Sherer TB, Betarbet R, Panov AV. Complex I and Parkinson’s Disease. IUBMB Life. 2001;52:135–41.
Su B, Wang X, Zheng L, Perry G, Smith MA, Zhu X. Abnormal mitochondrial dynamics and neurodegenerative diseases. Biochem Biophys Acta. 2010;1802:135–42.
Henchcliffe C, Beal MF. Mitochondrial biology and oxidative stress in Parkinson disease pathogenesis. Nat Clin Pract Neurol. 2008;4:600–9.
Lin MT, Beal MF. Mitochondrial dysfunction and oxidative stress in neurodegenerative diseases. Nature. 2006;443:787–95.
Bose A, Beal MF. Mitochondrial dysfunction in Parkinson’s disease. J Neurochem. 2016;139(Suppl 1):216–31.
Sulzer D, Zecca L. Intraneuronal dopamine-quinone synthesis: a review. Neurotox Res. 2000;1:181–95.
Mansur A, Comley R, Lewis Y, Middleton L, Huiban M, Guo Q, Passchier J, Tsukada H, Gunn R, Rabiner E. MIND MAPS CONSORTIUM ft: <strong>Imaging of Mitochondrial Complex 1 with <sup>18</sup>F-BCPP-EF in the Healthy Human Brain</strong>. J Nucl Med. 2018;59:1709–1709.
Berman DS, Maddahi J, Tamarappoo BK, Czernin J, Taillefer R, Udelson JE, Gibson CM, Devine M, Lazewatsky J, Bhat G, Washburn D. Phase II safety and clinical comparison with single-photon emission computed tomography myocardial perfusion imaging for detection of coronary artery disease: flurpiridaz F 18 positron emission tomography. J Am Coll Cardiol. 2013;61:469–77.
Bengs S, Warnock GI, Portmann A, Mikail N, Rossi A, Ahmed H, Etter D, Treyer V, Gisler L, Pfister SK, et al. Rest/stress myocardial perfusion imaging by positron emission tomography with (18)F-Flurpiridaz: A feasibility study in mice. J Nucl Cardiol. 2022. https://doi.org/10.1007/s12350-022-02968-9.
Haider A, Bengs S, Portmann A, Rossi A, Ahmed H, Etter D, Warnock GI, Mikail N, Grämer M, Meisel A, et al. Role of sex hormones in modulating myocardial perfusion and coronary flow reserve. Eur J Nucl Med Mol Imaging. 2022;49:2209–18.
Khan NL, Valente EM, Bentivoglio AR, Wood NW, Albanese A, Brooks DJ, Piccini P. Clinical and subclinical dopaminergic dysfunction in PARK6-linked parkinsonism: an 18F-dopa PET study. Ann Neurol. 2002;52:849–53.
Gan-Or Z, Liong C, Alcalay RN. GBA-Associated Parkinson’s Disease and Other Synucleinopathies. Curr Neurol Neurosci Rep. 2018;18:44.
Choi JH, Stubblefield B, Cookson MR, Goldin E, Velayati A, Tayebi N, Sidransky E. Aggregation of alpha-synuclein in brain samples from subjects with glucocerebrosidase mutations. Mol Genet Metab. 2011;104:185–8.
Mazzulli JR, Xu YH, Sun Y, Knight AL, McLean PJ, Caldwell GA, Sidransky E, Grabowski GA, Krainc D. Gaucher disease glucocerebrosidase and alpha-synuclein form a bidirectional pathogenic loop in synucleinopathies. Cell. 2011;146:37–52.
Migdalska-Richards A, Daly L, Bezard E, Schapira AH. Ambroxol effects in glucocerebrosidase and alpha-synuclein transgenic mice. Ann Neurol. 2016;80:766–75.
Zimprich A, Biskup S, Leitner P, Lichtner P, Farrer M, Lincoln S, Kachergus J, Hulihan M, Uitti RJ, Calne DB, et al. Mutations in LRRK2 cause autosomal-dominant parkinsonism with pleomorphic pathology. Neuron. 2004;44:601–7.
Chen J, Chen Y, Pu J. Leucine-Rich Repeat Kinase 2 in Parkinson’s Disease: Updated from Pathogenesis to Potential Therapeutic Target. Eur Neurol. 2018;79:256–65.
Chen Z, Shao T, Gao W, Fu H, Collier TL, Rong J, Deng X, Yu Q, Zhang X, Davenport AT, et al. Synthesis and Preliminary Evaluation of [(11) C]GNE-1023 as a Potent PET Probe for Imaging Leucine-Rich Repeat Kinase 2 (LRRK2) in Parkinson’s Disease. ChemMedChem. 2019;14:1580–5.
Malik N, Kornelsen R, McCormick S, Colpo N, Merkens H, Bendre S, Benard F, Sossi V, Schirrmacher R, Schaffer P. Development and biological evaluation of[(18)F]FMN3PA & [(18)F]FMN3PU for leucine-rich repeat kinase 2 (LRRK2) in vivo PET imaging. Eur J Med Chem. 2021;211:113005.
Rideout HJ, Chartier-Harlin MC, Fell MJ, Hirst WD, Huntwork-Rodriguez S, Leyns CEG, Mabrouk OS, Taymans JM. The Current State-of-the Art of LRRK2-Based Biomarker Assay Development in Parkinson’s Disease. Front Neurosci. 2020;14:865.
Chen Z, Chen J, Chen L, Yoo C-H, Rong J, Fu H, Shao T, Coffman K, Steyn SJ, Davenport AT, et al: Imaging Leucine-Rich Repeat Kinase 2 In Vivo with 18F-Labeled Positron Emission Tomography Ligand. J Med Chem. 2022.
Tolosa E, Vila M, Klein C, Rascol O. LRRK2 in Parkinson disease: challenges of clinical trials. Nat Rev Neurol. 2020;16:97–107.
Lin X, Parisiadou L, Gu XL, Wang L, Shim H, Sun L, Xie C, Long CX, Yang WJ, Ding J, et al. Leucine-rich repeat kinase 2 regulates the progression of neuropathology induced by Parkinson’s-disease-related mutant alpha-synuclein. Neuron. 2009;64:807–27.
Cookson MR. The role of leucine-rich repeat kinase 2 (LRRK2) in Parkinson’s disease. Nat Rev Neurosci. 2010;11:791–7.
Simunovic F, Yi M, Wang Y, Macey L, Brown LT, Krichevsky AM, Andersen SL, Stephens RM, Benes FM, Sonntag KC. Gene expression profiling of substantia nigra dopamine neurons: further insights into Parkinson’s disease pathology. Brain. 2009;132:1795–809.
Manzoni C, Lewis PA. LRRK2 and Autophagy. Adv Neurobiol. 2017;14:89–105.
Jennings D, Huntwork-Rodriguez S, Henry AG, Sasaki JC, Meisner R, Diaz D, Solanoy H, Wang X, Negrou E, Bondar VV, et al: Preclinical and clinical evaluation of the LRRK2 inhibitor DNL201 for Parkinson’s disease. Sci Transl Med. 2022;14:eabj2658.
West AB. Achieving neuroprotection with LRRK2 kinase inhibitors in Parkinson disease. Exp Neurol. 2017;298:236–45.
Zhao HT, John N, Delic V, Ikeda-Lee K, Kim A, Weihofen A, Swayze EE, Kordasiewicz HB, West AB, Volpicelli-Daley LA. LRRK2 Antisense Oligonucleotides Ameliorate alpha-Synuclein Inclusion Formation in a Parkinson’s Disease Mouse Model. Mol Therapy Nucleic Acids. 2017;8:508–19.
Ntetsika T, Papathoma PE, Markaki I. Novel targeted therapies for Parkinson’s disease. Mol Med. 2021;27:17.
Lee JY, Koga H, Kawaguchi Y, Tang W, Wong E, Gao YS, Pandey UB, Kaushik S, Tresse E, Lu J, et al. HDAC6 controls autophagosome maturation essential for ubiquitin-selective quality-control autophagy. Embo j. 2010;29:969–80.
Pandey UB, Nie Z, Batlevi Y, McCray BA, Ritson GP, Nedelsky NB, Schwartz SL, DiProspero NA, Knight MA, Schuldiner O, et al. HDAC6 rescues neurodegeneration and provides an essential link between autophagy and the UPS. Nature. 2007;447:860–4.
Simões-Pires C, Zwick V, Nurisso A, Schenker E, Carrupt PA, Cuendet M. HDAC6 as a target for neurodegenerative diseases: what makes it different from the other HDACs? Mol Neurodegener. 2013;8:7.
Koole M, Van Weehaeghe D, Serdons K, Herbots M, Cawthorne C, Celen S, Schroeder FA, Hooker JM, Bormans G, de Hoon J, et al. Clinical validation of the novel HDAC6 radiotracer [(18)F]EKZ-001 in the human brain. Eur J Nucl Med Mol Imaging. 2021;48:596–611.
Vila M, Jackson-Lewis V, Guegan C, Wu DC, Teismann P, Choi DK, Tieu K, Przedborski S. The role of glial cells in Parkinson’s disease. Curr Opin Neurol. 2001;14:483–9.
Kurkowska-Jastrzebska I, Wronska A, Kohutnicka M, Czlonkowski A, Czlonkowska A. The inflammatory reaction following 1-methyl-4-phenyl-1,2,3, 6-tetrahydropyridine intoxication in mouse. Exp Neurol. 1999;156:50–61.
Fu R, Shen Q, Xu P, Luo JJ, Tang Y. Phagocytosis of microglia in the central nervous system diseases. Mol Neurobiol. 2014;49:1422–34.
Nayak D, Roth TL, McGavern DB. Microglia development and function. Annu Rev Immunol. 2014;32:367–402.
Rupprecht R, Papadopoulos V, Rammes G, Baghai TC, Fan J, Akula N, Groyer G, Adams D, Schumacher M. Translocator protein (18 kDa) (TSPO) as a therapeutic target for neurological and psychiatric disorders. Nat Rev Drug Discovery. 2010;9:971–88.
Venneti S, Lopresti BJ, Wiley CA. The peripheral benzodiazepine receptor (Translocator protein 18kDa) in microglia: from pathology to imaging. Prog Neurobiol. 2006;80:308–22.
Brooks DJ. Technology Insight: imaging neurodegeneration in Parkinson’s disease. Nat Clin Pract Neurol. 2008;4:267–77.
Imamura K, Hishikawa N, Sawada M, Nagatsu T, Yoshida M, Hashizume Y. Distribution of major histocompatibility complex class II-positive microglia and cytokine profile of Parkinson’s disease brains. Acta Neuropathol. 2003;106:518–26.
Bartels AL, Willemsen AT, Doorduin J, de Vries EF, Dierckx RA, Leenders KL. [11C]-PK11195 PET: quantification of neuroinflammation and a monitor of anti-inflammatory treatment in Parkinson’s disease? Parkinsonism Relat Disord. 2010;16:57–9.
Gerhard A, Pavese N, Hotton G, Turkheimer F, Es M, Hammers A, Eggert K, Oertel W, Banati RB, Brooks DJ. In vivo imaging of microglial activation with [11C](R)-PK11195 PET in idiopathic Parkinson’s disease. Neurobiol Dis. 2006;21:404–12.
Ouchi Y, Yoshikawa E, Sekine Y, Futatsubashi M, Kanno T, Ogusu T, Torizuka T. Microglial activation and dopamine terminal loss in early Parkinson’s disease. Ann Neurol. 2005;57:168–75.
Kobylecki C, Counsell SJ, Cabanel N, Wächter T, Turkheimer FE, Eggert K, Oertel W, Brooks DJ, Gerhard A. Diffusion-weighted imaging and its relationship to microglial activation in parkinsonian syndromes. Parkinsonism Relat Disord. 2013;19:527–32.
Chauveau F, Van Camp N, Dolle F, Kuhnast B, Hinnen F, Damont A, Boutin H, James M, Kassiou M, Tavitian B. Comparative evaluation of the translocator protein radioligands 11C-DPA-713, 18F-DPA-714, and 11C-PK11195 in a rat model of acute neuroinflammation. J Nucl Med. 2009;50:468–76.
Zhang M-R, Maeda J, Furutsuka K, Yoshida Y, Ogawa M, Suhara T, Suzuki K. [18F]FMDAA1106 and [18F]FEDAA1106: two positron-Emitter labeled ligands for peripheral benzodiazepine receptor (PBR). Bioorg Med Chem Lett. 2003;13:201–4.
Zhang M-R, Kida T, Noguchi J, Furutsuka K, Maeda J, Suhara T, Suzuki K. [11C]DAA1106: radiosynthesis and in vivo binding to peripheral benzodiazepine receptors in mouse brain. Nucl Med Biol. 2003;30:513–9.
Maeda J, Suhara T, Zhang MR, Okauchi T, Yasuno F, Ikoma Y, Inaji M, Nagai Y, Takano A, Obayashi S, Suzuki K. Novel peripheral benzodiazepine receptor ligand [11C]DAA1106 for PET: an imaging tool for glial cells in the brain. Synapse. 2004;52:283–91.
Yui J, Hatori A, Kawamura K, Yanamoto K, Yamasaki T, Ogawa M, Yoshida Y, Kumata K, Fujinaga M, Nengaki N, et al. Visualization of early infarction in rat brain after ischemia using a translocator protein (18 kDa) PET ligand [11C]DAC with ultra-high specific activity. Neuroimage. 2011;54:123–30.
Gulyas B, Toth M, Vas A, Shchukin E, Kostulas K, Hillert J, Halldin C. Visualising neuroinflammation in post-stroke patients: a comparative PET study with the TSPO molecular imaging biomarkers [11C]PK11195 and [11C]vinpocetine. Curr Radiopharm. 2012;5:19–28.
Brody AL, Gehlbach D, Garcia LY, Enoki R, Hoh C, Vera D, Kotta KK, London ED, Okita K, Nurmi EL, et al. Effect of overnight smoking abstinence on a marker for microglial activation: a [(11)C]DAA1106 positron emission tomography study. Psychopharmacology. 2018;235:3525–34.
Best L, Ghadery C, Pavese N, Tai YF, Strafella AP. New and Old TSPO PET Radioligands for Imaging Brain Microglial Activation in Neurodegenerative Disease. Curr Neurol Neurosci Rep. 2019;19:24.
Zhang L, Hu K, Shao T, Hou L, Zhang S, Ye W, Josephson L, Meyer JH, Zhang MR, Vasdev N, et al. Recent developments on PET radiotracers for TSPO and their applications in neuroimaging. Acta pharmaceutica Sinica B. 2021;11:373–93.
Unterrainer M, Mahler C, Vomacka L, Lindner S, Havla J, Brendel M, Böning G, Ertl-Wagner B, Kümpfel T, Milenkovic VM, et al. TSPO PET with [18F]GE-180 sensitively detects focal neuroinflammation in patients with relapsing–remitting multiple sclerosis. Eur J Nucl Med Mol Imaging. 2018;45:1423–31.
Wadsworth H, Jones PA, Chau WF, Durrant C, Fouladi N, Passmore J, O’Shea D, Wynn D, Morisson-Iveson V, Ewan A, et al. [18F]GE-180: a novel fluorine-18 labelled PET tracer for imaging Translocator protein 18 kDa (TSPO). Bioorg Med Chem Lett. 2012;22:1308–13.
Alam MM, Lee J, Lee SY. Recent Progress in the Development of TSPO PET Ligands for Neuroinflammation Imaging in Neurological Diseases. Nucl Med Mol Imaging. 2017;51:283–96.
Ikawa M, Lohith TG, Shrestha S, Telu S, Zoghbi SS, Castellano S, Taliani S, Da Settimo F, Fujita M, Pike VW, Innis RB. 11C-ER176, a Radioligand for 18-kDa Translocator Protein, Has Adequate Sensitivity to Robustly Image All Three Affinity Genotypes in Human Brain. J Nucl Med. 2017;58:320–5.
Siméon FG, Lee J-H, Morse CL, Stukes I, Zoghbi SS, Manly LS, Liow J-S, Gladding RL, Dick RM, Yan X, et al. Synthesis and Screening in Mice of Fluorine-Containing PET Radioligands for TSPO: Discovery of a Promising 18F-Labeled Ligand. J Med Chem. 2021;64:16731–45.
Athauda D, Maclagan K, Skene SS, Bajwa-Joseph M, Letchford D, Chowdhury K, Hibbert S, Budnik N, Zampedri L, Dickson J, et al. Exenatide once weekly versus placebo in Parkinson’s disease: a randomised, double-blind, placebo-controlled trial. Lancet. 2017;390:1664–75.
Tansey MG, Wallings RL, Houser MC, Herrick MK, Keating CE, Joers V. Inflammation and immune dysfunction in Parkinson disease. Nat Rev Immunol. 2022;22(11):657–73.
Fujimoto H, Fujita N, Hamamatsu K, Murakami T, Nakamoto Y, Saga T, Ishimori T, Shimizu Y, Watanabe H, Sano K, et al. First-in-Human Evaluation of Positron Emission Tomography/Computed Tomography With [(18)F]FB(ePEG12)12-Exendin-4: A Phase 1 Clinical Study Targeting GLP-1 Receptor Expression Cells in Pancreas. Front Endocrinol (Lausanne). 2021;12:717101.
Luo Y, Pan Q, Yao S, Yu M, Wu W, Xue H, Kiesewetter DO, Zhu Z, Li F, Zhao Y, Chen X. Glucagon-Like Peptide-1 Receptor PET/CT with 68Ga-NOTA-Exendin-4 for Detecting Localized Insulinoma: A Prospective Cohort Study. J Nucl Med. 2016;57:715–20.
Antwi K, Fani M, Nicolas G, Rottenburger C, Heye T, Reubi JC, Gloor B, Christ E, Wild D. Localization of Hidden Insulinomas with 68Ga-DOTA-Exendin-4 PET/CT: A Pilot Study. J Nucl Med. 2015;56:1075–8.
Liu Y, Liu S, Liu L, Gong X, Liu J, Sun L, Liu X, Wu L, Chen L, Wang L, et al. Fine Comparison of the Efficacy and Safety Between GB242 and Infliximab in Patients with Rheumatoid Arthritis: A Phase III Study. Rheumatol Ther. 2022;9:175–89.
Tobinick EL, Chen K, Chen X. Rapid intracerebroventricular delivery of Cu-DOTA-etanercept after peripheral administration demonstrated by PET imaging. BMC Res Notes. 2009;2:28.
Colonna M. TREMs in the immune system and beyond. Nat Rev Immunol. 2003;3:445–53.
Guo Y, Wei X, Yan H, Qin Y, Yan S, Liu J, Zhao Y, Jiang F, Lou H. TREM2 deficiency aggravates α-synuclein-induced neurodegeneration and neuroinflammation in Parkinson’s disease models. Faseb j. 2019;33:12164–74.
Wilson EN, Swarovski MS, Linortner P, Shahid M, Zuckerman AJ, Wang Q, Channappa D, Minhas PS, Mhatre SD, Plowey ED, et al. Soluble TREM2 is elevated in Parkinson’s disease subgroups with increased CSF tau. Brain. 2020;143:932–43.
Meier SR, Sehlin D, Hultqvist G, Syvänen S. Pinpointing Brain TREM2 Levels in Two Mouse Models of Alzheimer’s Disease. Mol Imaging Biol. 2021;23:665–75.
Sanjari Moghaddam H, Zare-Shahabadi A, Rahmani F, Rezaei N. Neurotransmission systems in Parkinson’s disease. Rev Neurosci. 2017;28:509–36.
Tanimura A, Pancani T, Lim SAO, Tubert C, Melendez AE, Shen W, Surmeier DJ. Striatal cholinergic interneurons and Parkinson’s disease. Eur J Neurosci. 2018;47:1148–58.
Aosaki T, Miura M, Suzuki T, Nishimura K, Masuda M. Acetylcholine-dopamine balance hypothesis in the striatum: an update. Geriatr Gerontol Int. 2010;10(Suppl 1):S148-157.
Maurice N, Liberge M, Jaouen F, Ztaou S, Hanini M, Camon J, Deisseroth K, Amalric M, Kerkerian-Le Goff L, Beurrier C. Striatal Cholinergic Interneurons Control Motor Behavior and Basal Ganglia Function in Experimental Parkinsonism. Cell Rep. 2015;13:657–66.
Pisani A, Bernardi G, Ding J, Surmeier DJ. Re-emergence of striatal cholinergic interneurons in movement disorders. Trends Neurosci. 2007;30:545–53.
Ztaou S, Amalric M. Contribution of cholinergic interneurons to striatal pathophysiology in Parkinson’s disease. Neurochem Int. 2019;126:1–10.
DeMaagd G, Philip A. Parkinson’s Disease and Its Management: Part 3: Nondopaminergic and Nonpharmacological Treatment Options. P T. 2015;40:668–79.
Appenzeller O, Goss JE. Autonomic deficits in Parkinson’s syndrome. Arch Neurol. 1971;24:50–7.
Chen Z, Li G, Liu J. Autonomic dysfunction in Parkinson’s disease: Implications for pathophysiology, diagnosis, and treatment. Neurobiol Dis. 2020;134: 104700.
Orimo S, Uchihara T, Nakamura A, Mori F, Kakita A, Wakabayashi K, Takahashi H. Axonal alpha-synuclein aggregates herald centripetal degeneration of cardiac sympathetic nerve in Parkinson’s disease. Brain. 2008;131:642–50.
Phillips RJ, Walter GC, Wilder SL, Baronowsky EA, Powley TL. Alpha-synuclein-immunopositive myenteric neurons and vagal preganglionic terminals: autonomic pathway implicated in Parkinson’s disease? Neuroscience. 2008;153:733–50.
Goldstein DS, Sharabi Y, Karp BI, Bentho O, Saleem A, Pacak K, Eisenhofer G. Cardiac sympathetic denervation preceding motor signs in Parkinson disease. Clin Auton Res. 2007;17:118–21.
Goldstein DS. Dysautonomia in Parkinson’s disease: neurocardiological abnormalities. Lancet Neurol. 2003;2:669–76.
Treglia G, Cason E, Stefanelli A, Cocciolillo F, Di Giuda D, Fagioli G, Giordano A. MIBG scintigraphy in differential diagnosis of Parkinsonism: a meta-analysis. Clin Auton Res. 2012;22:43–55.
Beaulieu J-M, Espinoza S, Gainetdinov RR. Dopamine receptors – IUPHAR Review 13. Br J Pharmacol. 2015;172:1–23.
Seeman P, Niznik HB. Dopamine receptors and transporters in Parkinson’s disease and schizophrenia. FASEB J. 1990;4:2737–44.
Kaasinen V, Ruottinen HM, Någren K, Lehikoinen P, Oikonen V, Rinne JO. Upregulation of putaminal dopamine D2 receptors in early Parkinson’s disease: a comparative PET study with [11C] raclopride and [11C]N-methylspiperone. J Nucl Med. 2000;41:65–70.
Farde L, Halldin C, Stone-Elander S, Sedvall G. PET analysis of human dopamine receptor subtypes using 11C-SCH 23390 and 11C-raclopride. Psychopharmacology. 1987;92(3):278–84.
Halldin C, Farde L, Barnett A, Sedvall G. Synthesis of carbon-11 labelled SCH 39166, a new selective dopamine D-1 receptor ligand, and preliminary PET investigations. Int J Rad Appl Instrum A. 1991;42:451–5.
Halldin C, Foged C, Chou Y-H, Karlsson P, Swahn C-G, Johan S, Sedvall G, Farde L. Carbon-11-NNC 112: A Radioligand for PET Examination of Striatal and Neocortical D<sub>1</sub>-Dopamine Receptors. J Nucl Med. 1998;39:2061.
Ekelund J, Slifstein M, Narendran R, Guillin O, Belani H, Guo N-N, Hwang Y, Hwang D-R, Abi-Dargham A, Laruelle M. In Vivo DA D1 Receptor Selectivity of NNC 112 and SCH 23390. Mol Imag Biol. 2007;9:117–25.
Tamagnan G, Barret O, Alagille D, Carroll V, Madonia J, Constantinescu C, SanDiego C, Papin C, Morley T, Russell D, et al. T156. In vivo characterization of the first agonist dopamine D1 receptors PET imaging tracer [18F]MNI-968 in human. Schizophr Bull. 2018;44:1.
Ray NJ, Miyasaki JM, Zurowski M, Ko JH, Cho SS, Pellecchia G, Antonelli F, Houle S, Lang AE, Strafella AP. Extrastriatal dopaminergic abnormalities of DA homeostasis in Parkinson’s patients with medication-induced pathological gambling: a [11C] FLB-457 and PET study. Neurobiol Dis. 2012;48:519–25.
Nyberg S, Farde L, Eriksson L, Halldin C, Eriksson B. 5-HT2 and D2 dopamine receptor occupancy in the living human brain A PET study with risperidone. Psychopharmacology. 1993;110:265–72.
Chefer SI, Kimes AS, Matochik JA, Horti AG, Kurian V, Shumway D, Domino EF, London ED, Mukhin AG. Estimation of D2-like receptor occupancy by dopamine in the putamen of hemiparkinsonian Monkeys. Neuropsychopharmacology. 2008;33:270–8.
Sahin G, Thompson LH, Lavisse S, Ozgur M, Rbah-Vidal L, Dollé F, Hantraye P, Kirik D. Differential dopamine receptor occupancy underlies L-DOPA-induced dyskinesia in a rat model of Parkinson’s disease. PLoS ONE. 2014;9:e90759.
de la Fuente-Fernández R, Sossi V, Huang Z, Furtado S, Lu JQ, Calne DB, Ruth TJ, Stoessl AJ. Levodopa-induced changes in synaptic dopamine levels increase with progression of Parkinson’s disease: implications for dyskinesias. Brain. 2004;127:2747–54.
Del Bello F, Giannella M, Giorgioni G, Piergentili A, Quaglia W. Receptor Ligands as Helping Hands to L-DOPA in the Treatment of Parkinson’s Disease. Biomolecules. 2019;9(4):142.
Clarke CE, Guttman M. Dopamine agonist monotherapy in Parkinson’s disease. The Lancet. 2002;360:1767–9.
Blandini F, Armentero MT. Dopamine receptor agonists for Parkinson’s disease. Expert Opin Investig Drugs. 2014;23:387–410.
Davie CA. A review of Parkinson’s disease. Br Med Bull. 2008;86:109–27.
Abbott A. Levodopa: the story so far. Nature. 2010;466:S6–7.
Guggenheim M. Dioxyphenylalanin, eine neue Aminosäure aus Vicia faba. Biological Chemistry. 1913;88:276–84.
Holtz P. Dopadecarboxylase. Naturwissenschaften. 1939;27:724–5.
Hornykiewicz O. A brief history of levodopa. J Neurol. 2010;257:249–52.
Neurology TL. Building on 50 years of levodopa therapy. Lancet Neurol. 2016;15:1.
Senek M, Nielsen EI, Nyholm D. Levodopa-entacapone-carbidopa intestinal gel in Parkinson’s disease: A randomized crossover study. Mov Disord. 2017;32:283–6.
Antonini A. Levodopa in the treatment of Parkinson’s disease: an old drug still going strong. Clin Interv Aging. 2010;5:229.
Tambasco N, Romoli M, Calabresi P. Levodopa in Parkinson’s Disease: Current Status and Future Developments. Curr Neuropharmacol. 2018;16:1239–52.
Nutl JG, Fellman JH. Pharmacokinetics of Levodopa. Clin Neuropharmacol. 1984;7:35–50.
Hauser RA. Levodopa: Past, Present, and Future. Eur Neurol. 2009;62:1–8.
Cedarbaum JM. Clinical Pharmacokinetics of Anti-Parkinsonian Drugs. Clin Pharmacokinet. 1987;13:141–78.
Salat D, Tolosa E. Levodopa in the Treatment of Parkinson’s Disease: Current Status and New Developments. J Parkinson’s dis. 2013;3:255–69.
Lipp MM, Batycky R, Moore J, Leinonen M, Freed MI. Preclinical and clinical assessment of inhaled levodopa for OFF episodes in Parkinson’s disease. Sci Transl Med. 2016;8:360ra136.
Ellenbogen A, Stocchi F, Espay A, Poewe W, Oren S, Case R, Olanow CW. Impact of Subcutaneous Levodopa Infusion with ND0612 on Patient Reported Outcomes (4506). Neurology. 2020;94:4506.
Birnberg T, Smania G, Bjornsson M, Jonsson N, Case R, Oren S, Adar L, Karlsson M. Pharmacokinetic analysis of levodopa and carbidopa following subcutaneous infusion: A population pharmacokinetics model (2019). Neurology. 2019;2021:96.
Urso D, Chaudhuri KR, Qamar MA, Jenner P. Improving the Delivery of Levodopa in Parkinson’s Disease: A Review of Approved and Emerging Therapies. CNS Drugs. 2020;34:1149–63.
Müller T. Catechol-O-methyltransferase inhibitors in Parkinson’s disease. Drugs. 2015;75:157–74.
Ferreira JJ, Lees A, Rocha JF, Poewe W, Rascol O, Soares-da-Silva P. Opicapone as an adjunct to levodopa in patients with Parkinson’s disease and end-of-dose motor fluctuations: a randomised, double-blind, controlled trial. Lancet Neurol. 2016;15:154–65.
Schapira AH. Monoamine oxidase B inhibitors for the treatment of Parkinson’s disease: a review of symptomatic and potential disease-modifying effects. CNS Drugs. 2011;25:1061–71.
Fox SH, Katzenschlager R, Lim S-Y, Ravina B, Seppi K, Coelho M, Poewe W, Rascol O, Goetz CG, Sampaio C. The Movement Disorder Society Evidence-Based Medicine Review Update: Treatments for the motor symptoms of Parkinson’s disease. Mov Disord. 2011;26:S2–41.
Group PS. A controlled trial of rasagiline in early Parkinson disease: the TEMPO Study. Arch Neurol. 2002;59:1937–43.
Schapira AH, Fox SH, Hauser RA, Jankovic J, Jost WH, Kenney C, Kulisevsky J, Pahwa R, Poewe W, Anand R. Assessment of Safety and Efficacy of Safinamide as a Levodopa Adjunct in Patients With Parkinson Disease and Motor Fluctuations: A Randomized Clinical Trial. JAMA Neurol. 2017;74:216–24.
Ellis JM, Fell MJ. Current approaches to the treatment of Parkinson’s Disease. Bioorg Med Chem Lett. 2017;27:4247–55.
Bezard E, Brotchie JM, Gross CE. Pathophysiology of levodopa-induced dyskinesia: Potential for new therapies. Nat Rev Neurosci. 2001;2:577–88.
Lee J, Zhu W-M, Stanic D, Finkelstein DI, Horne MH, Henderson J, Lawrence AJ, O’Connor L, Tomas D, Drago J, Horne MK. Sprouting of dopamine terminals and altered dopamine release and uptake in Parkinsonian dyskinaesia. Brain. 2008;131:1574–87.
Olanow CW, Obeso JA, Stocchi F. Continuous dopamine-receptor treatment of Parkinson’s disease: scientific rationale and clinical implications. Lancet Neurol. 2006;5:677–87.
Antonini A, Fung VS, Boyd JT, Slevin JT, Hall C, Chatamra K, Eaton S, Benesh JA. Effect of levodopa-carbidopa intestinal gel on dyskinesia in advanced Parkinson’s disease patients. Mov Disord. 2016;31:530–7.
Poewe W, Antonini A. Novel formulations and modes of delivery of levodopa. Mov Disord. 2015;30:114–20.
Firnau G, Nahmias C, Garnett S. The preparation of [18F]5-fluoro-DOPA with reactor-produced fluorine-18. Int J Appl Radiat Isot. 1973;24:182–4.
Garnett S, Firnau G, Nahmias C, Chirakal R. Striatal dopamine metabolism in living monkeys examined by positron emission tomography. Brain Res. 1983;280:169–71.
Nanni C, Fanti S, Rubello D. 18F-DOPA PET and PET/CT. J Nucl Med. 2007;48:1577–9.
Ribeiro M-J, Vidailhet M, Loc’h C, Dupel C, Nguyen JP, Ponchant M, Dollé F, Peschanski M, Hantraye P, Cesaro P, et al. Dopaminergic Function and Dopamine Transporter Binding Assessed With Positron Emission Tomography in Parkinson Disease. Arch Neurol. 2002;59:580–6.
Weeks RA, Brooks DJ. Positron emission tomography and central neurotransmitter systems in movement disorders. Fundam Clin Pharmacol. 1994;8:503–17.
Garnett ES, Firnau G, Nahmias C. Dopamine visualized in the basal ganglia of living man. Nature. 1983;305:137–8.
Piel M, Vernaleken I, Rosch F. Positron emission tomography in CNS drug discovery and drug monitoring. J Med Chem. 2014;57:9232–58.
Dhawan V, Niethammer M, Lesser M, Pappas K, Hellman M, Fitzpatrick T, Quartarolo L, Bjelke D, Eidelberg D, Chlay T. Prospective FDOPA PET imaging study in human PD :our final step towards NDA approval. J Nucl Med. 2020;61:1565.
Dannals RF, Neumeyer JL, Milius RA, Ravert HT, Wilson AA, Wagner HN Jr. Synthesis of a radiotracer for studying dopamine uptake sites in vivo using PET: 2β-carbomethoxy-3β-(4-fluorophenyl)-[N-11C-methyl]tropane ([11C]CFT or [11C]WIN-35,428). J Labelled Compd Radiopharm. 1993;33:147–52.
Rinne JO, Bergman J, Ruottinen H, Haaparanta M, Eronen E, Oikonen V, Sonninen P, Solin O. Striatal uptake of a novel PET ligand, [18F]beta-CFT, is reduced in early Parkinson’s disease. Synapse. 1999;31:119–24.
Appel L, Jonasson M, Danfors T, Nyholm D, Askmark H, Lubberink M, Sörensen J. Use of 11C-PE2I PET in differential diagnosis of parkinsonian disorders. J Nucl Med. 2015;56:234–42.
Fischman AJ, Bonab AA, Babich JW, Livni E, Alpert NM, Meltzer PC, Madras BK. [(11)C, (127)I] Altropane: a highly selective ligand for PET imaging of dopamine transporter sites. Synapse. 2001;39:332–42.
Seifert KD, Wiener JI. The impact of DaTscan on the diagnosis and management of movement disorders: A retrospective study. Am J Neurodegener Dis. 2013;2:29–34.
Frost JJ, Rosier AJ, Reich SG, Smith JS, Ehlers MD, Snyder SH, Ravert HT, Dannals RF. Positron emission tomographic imaging of the dopamine transporter with 11C-WIN 35,428 reveals marked declines in mild Parkinson’s disease. Ann Neurol. 1993;34:423–31.
Piccini PP. Dopamine transporter: Basic aspects and neuroimaging. Mov Disord. 2003;18:S3–8.
Brooks DJ. Molecular imaging of dopamine transporters. Ageing Res Rev. 2016;30:114–21.
Peter D, Liu Y, Sternini C, De Giorgio R, Brecha N, Edwards R. Differential expression of two vesicular monoamine transporters. J Neurosci. 1995;15:6179–88.
Vander Borght TM, Sima AAF, Kilbourn MR, Desmond TJ, Kuhl DE, Frey KA. [3H]methoxytetrabenazine: A high specific activity ligand for estimating monoaminergic neuronal integrity. Neuroscience. 1995;68:955–62.
Frey KA, Koeppe RA, Kilbourn MR, Vander Borght TM, Albin RL, Gilman S, Kuhl DE. Presynaptic monoaminergic vesicles in Parkinson’s disease and normal aging. Ann Neurol. 1996;40:873–84.
Okamura N, Villemagne VL, Drago J, Pejoska S, Dhamija RK, Mulligan RS, Ellis JR, Ackermann U, O’Keefe G, Jones G, et al. In vivo measurement of vesicular monoamine transporter type 2 density in Parkinson disease with (18)F-AV-133. J Nucl Med. 2010;51:223–8.
Hsiao IT, Weng YH, Hsieh CJ, Lin WY, Wey SP, Kung MP, Yen TC, Lu CS, Lin KJ. Correlation of Parkinson disease severity and 18F-DTBZ positron emission tomography. JAMA Neurol. 2014;71:758–66.
Chaudhuri KR, Healy DG, Schapira AH. Non-motor symptoms of Parkinson’s disease: diagnosis and management. Lancet Neurol. 2006;5:235–45.
Politis M, Wu K, Loane C, Kiferle L, Molloy S, Brooks DJ, Piccini P. Staging of serotonergic dysfunction in Parkinson’s disease: an in vivo 11C-DASB PET study. Neurobiol Dis. 2010;40:216–21.
Doder M, Rabiner EA, Turjanski N, Lees AJ, Brooks DJ. study CWP: Tremor in Parkinson’s disease and serotonergic dysfunction: an 11C-WAY 100635 PET study. Neurology. 2003;60:601–5.
Fu H, Rong J, Chen Z, Zhou J, Collier T, Liang SH. Positron Emission Tomography (PET) Imaging Tracers for Serotonin Receptors. J Med Chem. 2022;65:10755–808.
Wilson H, Dervenoulas G, Pagano G, Koros C, Yousaf T, Picillo M, Polychronis S, Simitsi A, Giordano B, Chappell Z, et al. Serotonergic pathology and disease burden in the premotor and motor phase of A53T alpha-synuclein parkinsonism: a cross-sectional study. Lancet Neurol. 2019;18:748–59.
Sahli ZT, Tarazi FI. Pimavanserin: novel pharmacotherapy for Parkinson’s disease psychosis. Expert Opin Drug Discov. 2018;13:103–10.
Johnson KA, Conn PJ, Niswender CM. Glutamate receptors as therapeutic targets for Parkinson’s disease. CNS Neurol Disord: Drug Targets. 2009;8:475–91.
Crupi R, Impellizzeri D, Cuzzocrea S. Role of Metabotropic Glutamate Receptors in Neurological Disorders. Front Mol Neurosci. 2019;12:20.
Karcz-Kubicha M, Lorenz B, Danysz W. GlycineB antagonists and partial agonists in rodent models of Parkinson’s disease–comparison with uncompetitive N-methyl-D-aspartate receptor antagonist. Neuropharmacology. 1999;38:109–19.
Ossowska K. The role of excitatory amino acids in models of Parkinson’s disease. J Neural Transm Park Dis Dement Sect. 1994;8:39–71.
Marino MJ, Valenti O, Conn PJ. Glutamate receptors and Parkinson’s disease: opportunities for intervention. Drugs Aging. 2003;20:377–97.
Marin C, Papa S, Engber TM, Bonastre M, Tolosa E, Chase TN. MK-801 prevents levodopa-induced motor response alterations in parkinsonian rats. Brain Res. 1996;736:202–5.
Sawada H, Oeda T, Kuno S, Nomoto M, Yamamoto K, Yamamoto M, Hisanaga K, Kawamura T, Amantadine Study G. Amantadine for dyskinesias in Parkinson’s disease: a randomized controlled trial. PLoS ONE. 2010;5:e15298.
Paoletti P, Neyton J. NMDA receptor subunits: function and pharmacology. Curr Opin Pharmacol. 2007;7:39–47.
Nash JE, Fox SH, Henry B, Hill MP, Peggs D, McGuire S, Maneuf Y, Hille C, Brotchie JM, Crossman AR. Antiparkinsonian actions of ifenprodil in the MPTP-lesioned marmoset model of Parkinson’s disease. Exp Neurol. 2000;165:136–42.
Steece-Collier K, Chambers LK, Jaw-Tsai SS, Menniti FS, Greenamyre JT. Antiparkinsonian actions of CP-101,606, an antagonist of NR2B subunit-containing N-methyl-d-aspartate receptors. Exp Neurol. 2000;163:239–43.
Smart K, Zheng MQ, Ahmed H, Fang H, Xu Y, Cai L, Holden D, Kapinos M, Haider A, Felchner Z, et al. Comparison of three novel radiotracers for GluN2B-containing NMDA receptors in non-human primates: (R)-[(11)C]NR2B-Me, (R)-[(18)F]of-Me-NB1, and (S)-[(18)F]of-NB1. J Cereb Blood Flow Metab. 2022;42:1398–409.
Ahmed H, Zheng MQ, Smart K, Fang H, Zhang L, Emery PR, Gao H, Ropchan J, Haider A, Tamagnan G, et al. Evaluation of (rac)-, (R)- and (S)-(18)F-OF-NB1 for imaging GluN2B subunit-containing N-methyl-D-aspartate receptors in non-human primates. J Nucl Med. 2022;63(12):1912–8.
Zheng M, Ahmed H, Smart K, Xu Y, Holden D, Kapinos M, Felchner Z, Haider A, Tamagnan G, Carson RE, et al. Characterization in nonhuman primates of (R)-[(18)F]OF-Me-NB1 and (S)-[(18)F]OF-Me-NB1 for imaging the GluN2B subunits of the NMDA receptor. Eur J Nucl Med Mol Imaging. 2022;49:2153–62.
Rischka L, Vraka C, Pichler V, Rasul S, Nics L, Gryglewski G, Handschuh P, Murgaš M, Godbersen GM, Silberbauer LR, et al. First-in-Humans Brain PET Imaging of the GluN2B-Containing N-methyl-d-aspartate Receptor with (R)-(11)C-Me-NB1. J Nucl Med. 2022;63:936–41.
Ahmed H, Wallimann R, Haider A, Hosseini V, Gruber S, Robledo M, Nguyen TAN, Herde AM, Iten I, Keller C, et al. Preclinical Development of (18)F-OF-NB1 for Imaging GluN2B-Containing N-Methyl-d-Aspartate Receptors and Its Utility as a Biomarker for Amyotrophic Lateral Sclerosis. J Nucl Med. 2021;62:259–65.
Ahmed H, Haider A, Varisco J, Stanković M, Wallimann R, Gruber S, Iten I, Häne S, Müller Herde A, Keller C, et al. Structure-Affinity Relationships of 2,3,4,5-Tetrahydro-1H-3-benzazepine and 6,7,8,9-Tetrahydro-5H-benzo[7]annulen-7-amine Analogues and the Discovery of a Radiofluorinated 2,3,4,5-Tetrahydro-1H-3-benzazepine Congener for Imaging GluN2B Subunit-Containing N-Methyl-d-aspartate Receptors. J Med Chem. 2019;62:9450–70.
Haider A, Iten I, Ahmed H, Müller Herder A, Gruber S, Krämer SD, Keller C, Schibli R, Wünsch B, Mu L, Ametamey SM. Identification and Preclinical Evaluation of a Radiofluorinated Benzazepine Derivative for Imaging the GluN2B Subunit of the Ionotropic NMDA Receptor. J Nucl Med. 2018;60:259–66.
Szermerski M, Börgel F, Schepmann D, Haider A, Betzel T, Ametamey SM, Wünsch B. Fluorinated GluN2B Receptor Antagonists with a 3-Benzazepine Scaffold Designed for PET Studies. ChemMedChem. 2018;13:1058–68.
Krämer SD, Betzel T, Mu L, Haider A, Herde AM, Boninsegni AK, Keller C, Szermerski M, Schibli R, Wünsch B, Ametamey SM. Evaluation of (11)C-Me-NB1 as a Potential PET Radioligand for Measuring GluN2B-Containing NMDA Receptors, Drug Occupancy, and Receptor Cross Talk. J Nucl Med. 2018;59:698–703.
Goldstein DS, Holmes C, Cannon RO, Eisenhofer G, Kopin IJ. Sympathetic Cardioneuropathy in Dysautonomias. N Engl J Med. 1997;336:696–702.
Sakakibara R, Tateno F, Kishi M, Tsuyusaki Y, Terada H, Inaoka T. MIBG myocardial scintigraphy in pre-motor Parkinson’s disease: A review. Parkinsonism Relat Disord. 2014;20:267–73.
De Pablo-Fernandez E, Tur C, Revesz T, Lees AJ, Holton JL, Warner TT. Association of Autonomic Dysfunction With Disease Progression and Survival in Parkinson Disease. JAMA Neurol. 2017;74:970–6.
De Pablo-Fernandez E, Warner TT. Autonomic Dysfunction in Parkinson’s Disease: The Hidden Game Changer? Mov Disord. 2018;33:1028.
Hauser RA, Heritier S, Rowse GJ, Hewitt LA, Isaacson SH. Droxidopa and Reduced Falls in a Trial of Parkinson Disease Patients With Neurogenic Orthostatic Hypotension. Clin Neuropharmacol. 2016;39:220–6.
Braune S, Reinhardt M, Schnitzer R, Riedel A, Lücking CH. Cardiac uptake of [123I]MIBG separates Parkinson’s disease from multiple system atrophy. Neurology. 1999;53:1020–5.
Rascol O, Schelosky L. 123I-metaiodobenzylguanidine scintigraphy in Parkinson’s disease and related disorders. Mov Disord. 2009;24(Suppl 2):S732-741.
Sources 2/ https://molecularneurodegeneration.biomedcentral.com/articles/10.1186/s13024-023-00600-z The mention sources can contact us to remove/changing this article |
What Are The Main Benefits Of Comparing Car Insurance Quotes Online
LOS ANGELES, CA / ACCESSWIRE / June 24, 2020, / Compare-autoinsurance.Org has launched a new blog post that presents the main benefits of comparing multiple car insurance quotes. For more info and free online quotes, please visit https://compare-autoinsurance.Org/the-advantages-of-comparing-prices-with-car-insurance-quotes-online/ The modern society has numerous technological advantages. One important advantage is the speed at which information is sent and received. With the help of the internet, the shopping habits of many persons have drastically changed. The car insurance industry hasn't remained untouched by these changes. On the internet, drivers can compare insurance prices and find out which sellers have the best offers. View photos The advantages of comparing online car insurance quotes are the following: Online quotes can be obtained from anywhere and at any time. Unlike physical insurance agencies, websites don't have a specific schedule and they are available at any time. Drivers that have busy working schedules, can compare quotes from anywhere and at any time, even at midnight. Multiple choices. Almost all insurance providers, no matter if they are well-known brands or just local insurers, have an online presence. Online quotes will allow policyholders the chance to discover multiple insurance companies and check their prices. Drivers are no longer required to get quotes from just a few known insurance companies. Also, local and regional insurers can provide lower insurance rates for the same services. Accurate insurance estimates. Online quotes can only be accurate if the customers provide accurate and real info about their car models and driving history. Lying about past driving incidents can make the price estimates to be lower, but when dealing with an insurance company lying to them is useless. Usually, insurance companies will do research about a potential customer before granting him coverage. Online quotes can be sorted easily. Although drivers are recommended to not choose a policy just based on its price, drivers can easily sort quotes by insurance price. Using brokerage websites will allow drivers to get quotes from multiple insurers, thus making the comparison faster and easier. For additional info, money-saving tips, and free car insurance quotes, visit https://compare-autoinsurance.Org/ Compare-autoinsurance.Org is an online provider of life, home, health, and auto insurance quotes. This website is unique because it does not simply stick to one kind of insurance provider, but brings the clients the best deals from many different online insurance carriers. In this way, clients have access to offers from multiple carriers all in one place: this website. On this site, customers have access to quotes for insurance plans from various agencies, such as local or nationwide agencies, brand names insurance companies, etc. "Online quotes can easily help drivers obtain better car insurance deals. All they have to do is to complete an online form with accurate and real info, then compare prices", said Russell Rabichev, Marketing Director of Internet Marketing Company. CONTACT: Company Name: Internet Marketing CompanyPerson for contact Name: Gurgu CPhone Number: (818) 359-3898Email: [email protected]: https://compare-autoinsurance.Org/ SOURCE: Compare-autoinsurance.Org View source version on accesswire.Com:https://www.Accesswire.Com/595055/What-Are-The-Main-Benefits-Of-Comparing-Car-Insurance-Quotes-Online View photos
to request, modification Contact us at Here or [email protected]